Novel Applications for Ultrafast Lasers in BME
The precise ablation that is possible with pico- and femtosecond lasers makes them ideal candidates for high-precision surgery. Routine procedures mostly revolve around the eye and include flap-cutting for Laser Assisted In-Situ Keratomileusis (LASIK)1 or creating precisely shaped corneal buttons for Keratoplasty procedures2. However, recently there has been increased interest in expanding the range of surgical applications for ultrafast laser systems.
Hard Tissue Manipulation
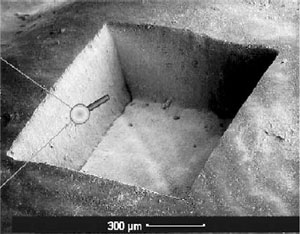
Figure 1. A 1 square millimeter area of human tooth enamel has been precisely ablated using femtosecond laser pulses. To create the square, the beam is scanned across the tissue layer by layer.
While a surgical laser system is certainly capable of cutting more precisely than a traditional bone saw, one of the shortcomings of fast-pulsed laser systems is their inability to remove large volumes of tissue in a reasonable amount of time, making them less than ideally suited for cutting through large bones like the femor. However, in dentistry, small volumes of hard tissue, namely small portions of teeth, have to be removed as part of routine surgeries.
The conventional approach involves a rapidly spinning drill that mechanically removes tissue. While this is effective, it is also unpleasant for the patient due to the high-frequency vibrations. Furthermore, this technique is relatively imprecise, because in order to ensure complete removal of dead tissue, dentists have to remove part of the healthy enamel as well. This creates larger cavities than necessary. In an effort to facilitate a less unpleasant and more precise removal of dental caries, lasers have been employed in dentistry to ablate cariotic tissue rather than using mechanical forces to remove it. These systems typically emit pulses in the range of several dozen nanoseconds, such as the Er:YAG system developed by VersaWave, Inc.
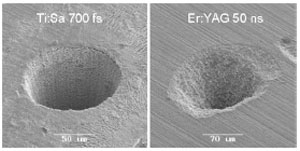
Figure 2. The effect of shorter pulses can be clearly seen in this picture comparing an ultrashort femtosecond pulse (left) to a nanosecond pulse induced ablation. Note the well-defined borders of the ablation volume on the left.
Replacing the mechanical drill with picosecond lasers has shown promising results3 and allowed for extremely precise removal of tissue (Figure 1)4, however the cavitation bubbles that form after LIOB tended to create microfractures in the enamel, weakening the tooth and creating a larger surface area for future infections. A relationship between pulse length and precision of the associated ablation has been established, in that shorter pulses allow for more precise cuts (Figure 2)4. By using shorter femtosecond pulses, photodisruption occurs in a more controlled manner, vastly reducing the amount of microfractures4. Laser systems eliminate the unpleasant vibrations and may help to reduce the apprehension that many patients feel when having to go to the dentist. Additionally, by combining spectral analysis of the plasma that the laser creates, it is possible to automate the removal process to the point where a sensor is able to distinguish between healthy and diseased tissues5 and ablate selectively6 rather than indiscriminately destroying healthy along with cariotic tissue.
Nanosurgery
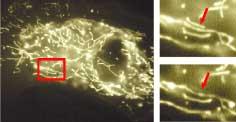
Figure 3. Elimination of an individual mitochondrion using femtosecond pulses. Despite its proximity to other mitochondria, only the targeted mitochondrion is eliminated (arrows)8.
Due to the highly constrained, micrometer-scale focal volume and the extreme brevity of the pulse that minimizes thermal effects in the area surrounding the target volume, femtosecond laser pulses can be used to selectively eliminate cellular components in individual cells, making them a powerful research tool. Shimada et al report on the elimination of individual mitochondria in living cells7 (Figure 3). Mitochondria serve as cellular powerplants and thus play an extremely important role in cellular functionality. Therefore, mitochondral abnormalities can have highly adverse effects on the health of entire organism. Studying these abnormalities in controlled conditions requires selective manipulation of mitochondria, which is difficult to achieve using chemical methods as these methods usually work in a statistic manner, i.e. it is usually not possible to predict exactly which or how many targets will be affected. Using fs lasers, abnormalities can be induced in cell culture by selectively eliminating mitochondria while retaining cellular viability. Remarkably, cells were shown to successfully proliferate even post-ablation.
Nanoscissors and Nanodissection
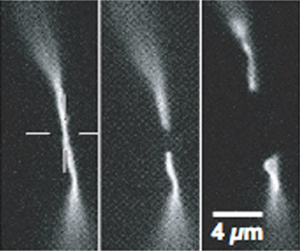
Figure 4. Cutting of individual actin stress fibers using femtosecond nanoscissors10.
Further application of fs pulses as nanoscissors allows for the study of cytoskeletal mechanisms by cutting fluorescent-labelled actin filaments and watching their subsequent retraction and polymerization, as reported by Shen et al8. Using 200fs pulses with a repetition rate of 1 kHz, single microtubule fibers could be cut and their recoil and depolimerization was observed9. Figure 4 shows work performed by Kumar et al, who were able to observe changes in cell shape as a result of cutting individual actin stress fibers using a similar technique10. In combination with chemically blocking molecular motors they found that cells actively exert force on these actin fibers in addition to their inherent passive elasticity.
Nanoscissors can also be used for nanodissection of chromosomes, as reported by König et al. By scanning the beam in a single line, they managed to successfully dissect human chromosomes inside a living cell while retaining cell viability 48 hours after laser exposure.11. Because multiphoton imaging can be achieved using the same laser pulses, albeit at lower power levels, it is possible to observe the cells in near real-time in vitro before, during and after laser nanodissection.
Nanodrills
A prerequisite for certain gene therapies is targeted transfection with foreign genetic material of specific cells within a cluster. This has been realized mechanically by using microneedles to achieve targeted transfection as well as electrically through electroporation and chemically using plasma-membrane permeabilization. Direct transfer through micromechanical injection using microneedles causes comparatively large holes in the cell membrane. Some plant cells have hard cell walls, causing the microneedle to break. There have also been problems with plasmid injection, and this technique is restricted to adherent cells. Being a contact method, it cannot be performed on cells inside a 3-D cluster.
Tsukakoshi et al successfully introduced a laser-based contact-free transfection method in 198412, however their transfection success rates remained low due to their use of comparatively long nanosecond pulses that caused excessive damage to the cells. The introduction of femtosecond laser systems for targeted transfection has yielded promising results13, allowing for contact-free, highly specific transfection of cells even within clusters while causing minimal damage to the cell walls.